Nous nous sommes installés à l’IBPC au sein de l’UMR 8261 en octobre 2016 pour développer une équipe de biologie structurale centrée sur l’étude des ARN. Nos compétences sont axées sur la biochimie des protéines et des ARN ainsi que sur la biologie structurale et la biophysique (RMN, cristallographie des rayons X, cryoEM, modélisation, microcalorimétrie, fluorescence). Nous utilisons et développons différentes méthodes de biologie structurale, notamment la spectroscopie RMN, afin d’étudier la biogenèse, l’architecture et les interactions des ARNs avec divers partenaires, ceci leur permettant d’accomplir leurs différentes fonctions. En effet, les ARN sont des acteurs majeurs de nombreux processus biologiques qui comprennent souvent une ou plusieurs étapes essentielles au cours de laquelle l'ARN subit des réarrangements structuraux impliquant, par exemple, la coupure d’un intron, l’incorporation de modifications post-transcriptionnelles ou plus globalement la modification des interactions secondaires ou tertiaires consécutives à la liaison d'un ligand (petite molécule, protéine ou un autre ARN). Ces changements structuraux vont finalement agir sur la fonction cellulaire. L’étude de ces changements structuraux motive nos travaux. Les projets que nous développons actuellement concernent principalement l’étude de protéines chaperonnes des ARNs et l’étude de la maturation des ARNs (ARNt et ARNr). Nos projets bénéficient de l’installation en juin 2016 d’un spectromètre RMN Bruker 700 MHz (Equipex CACSICE) et d’une cryosonde opérationnelle depuis janvier 2018.
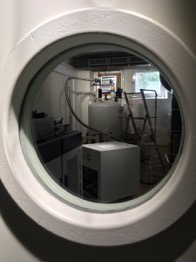
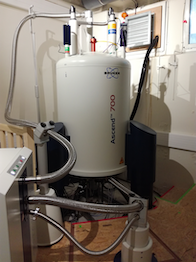
Plateforme RMN de l’IBPC
(Responsable scientifique P. Barraud)
S. Oerum, T. Dendooven, M. Catala, L. Gilet, C. Dégut, A. Trinquier, M. Bourguet, P. Barraud, S. Cianferani, B. F. Luisi, C. Condon*, C. Tisné* (2020). Structures of B. subtilis maturation RNases captured on 50S ribosome with pre-rRNAs, Mol Cell, doi: 10.1016/j.molcel.2020.09.008
Abstract:
The pathways for ribosomal RNA (rRNA) maturation diverge greatly among the domains of life. In the Gram- positive model bacterium, Bacillus subtilis, the final maturation steps of the two large ribosomal subunit (50S) rRNAs, 23S and 5S pre-rRNAs, are catalyzed by the double-strand specific ribonucleases (RNases) Mini- RNase III and RNase M5, respectively. Here we present a protocol that allowed us to solve the 3.0 and 3.1 A ̊ resolution cryoelectron microscopy structures of these RNases poised to cleave their pre-rRNA substrates within the B. subtilis 50S particle. These data provide the first structural insights into rRNA maturation in bacteria by revealing how these RNases recognize and process double-stranded pre-rRNA. Our structures further uncover how specific ribosomal proteins act as chaperones to correctly fold the pre-rRNA substrates and, for Mini-III, anchor the RNase to the ribosome. These r-proteins thereby serve a quality-control function in the process from accurate ribosome assembly to rRNA processing.
P. Barraud*, A. Gato, M. Heiss, M. Catala, S. Kellner & C. Tisné*, Time-resolved NMR monitoring of RNA maturation in cellular extracts revealed complex circuits of modifications in tRNAs, Nature Communications (2019), doi: 10.1038/s41467-019-11356-w
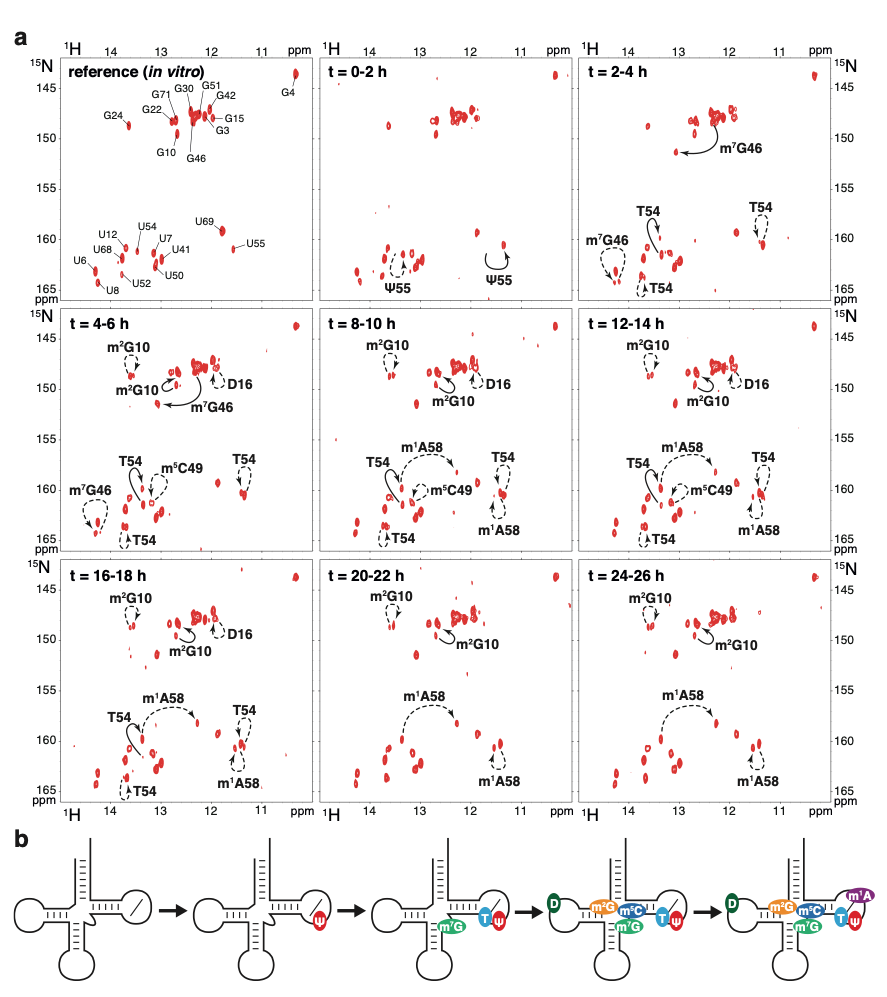
Abstract:
Although the biological importance of post-transcriptional RNA modifications in gene expression is widely appreciated, methods to directly detect the introduction of these modifications during RNA biosynthesis are rare and do not easily provide information on the temporal nature of events. Here we introduce the application of NMR spectroscopy to observe the maturation of tRNAs in cell extracts. By following the maturation of yeast tRNAPhe with time-resolved NMR measurements, we found that modifications are introduced in a defined sequential order, and that the chronology is controlled by cross-talk between modification events. In particular, we uncovered a strong hierarchy in the introduction of the T54, Ψ55 and m1A58 modifications in the T-arm, and demonstrate that the modification circuits identified in yeast extract with NMR also impact the tRNA modification process in living cells. The NMR-based methodology presented here could be adapted to investigate different aspects of tRNA maturation and RNA modifications in general.
C. Dégut#, M. Roovers#, P. Barraud, F. Brachet, A. Feller, V. Larue, A. Al Refaii, J. Caillet, L. Droogmans*, C. Tisné*, Structural characterisation of B. subtilis m1A22 tRNA methyltransferase TrmK: Insights into tRNA recognition, Nucleic Acids Research (2019), doi: 10.1093/nar/gkz230
Abstract:
1-Methyladenosine (m1 A) is a modified nucleoside found at positions 9, 14, 22 and 58 of tRNAs, which arises from the transfer of a methyl group onto the N1-atom of adenosine. The yqfN gene of Bacillus subtilis encodes the methyltransferase TrmK (BsTrmK) responsible for the formation of m1A22 in tRNA. Here, we show that BsTrmK displays a broad substrate specificity, and methylates seven out of eight tRNA isoacceptor families of B. subtilis bearing an A22. In addition to a non-Watson–Crick base-pair between the target A22 and a purine at position 13, the formation of m1 A22 by BsTrmK requires a full-length tRNA with intact tRNA elbow and anticodon stem. We solved the crystal structure of BsTrmK showing an N-terminal catalytic domain harbouring the typical Rossmann-like fold of Class-I methyltransferases and a C-terminal coiled-coil domain. We used NMR chemical shift mapping to drive the docking of BstRNASer to BsTrmK in complex with its methyl-donor cofactor S-adenosyl-L-methionine (SAM). In this model, validated by methyltransferase activity assays on BsTrmK mutants, both domains of BsTrmK participate in tRNA binding. BsTrmK recognises tRNA with very few structural changes in both partner, the non-Watson–Crick R13–A22 base-pair positioning the A22 N1-atom close to the SAM methyl group. P. Barraud* & C. Tisné, To be or not to be modified: miscellaneous aspects influencing nucleotide modifications in tRNAs,
Abstract:
Transfer RNAs (tRNAs) are essential components of the cellular protein synthesis machineries, but are also implicated in many roles outside translation. To become functional, tRNAs, initially transcribed as longer precursor tRNAs, undergo a tightly controlled biogenesis process comprising the maturation of their extremities, removal of intronic sequences if present, addition of the 30 -CCA amino-acid accepting sequence, and aminoacylation. In addition, the most impressive feature of tRNA biogenesis consists in the incorporation of a large number of post-transcriptional chemical modifications along its sequence. The chemical nature of these modifications is highly diverse, with more than hundred different modifications identified in tRNAs to date. All functions of tRNAs in cells are controlled and modulated by modifications, making the understanding of the mechanisms that determine and influence nucleotide modifications in tRNAs an essential point in tRNA biology. This review describes the different aspects that determine whether a certain position in a tRNA molecule is modified or not. We describe how sequence and structural determinants, as well as the presence of prior modifications control modification processes. We also describe how environmental factors and cellular stresses influence the level and/or the nature of certain modifications introduced in tRNAs, and report situations where these dynamic modulations of tRNA modification levels are regulated by active demodification processes.
S. Oerum#, M. Catala#, C. Atdjian, F. Brachet, L. Ponchon, P. Barraud, L. Iannazzo, L. Droogmans, E. Braud, M. Ethève-Quelquejeu & C. Tisné*, Bisubstrate analogues as structural tools to investigate m6A methyltransferase active sites,
RNA methyltransferases (MTases) catalyse the transfer of a methyl group to their RNA substrates using most- often S-adenosyl-L-methionine (SAM) as cofactor. Only few RNA-bound MTases structures are currently available due to the difficulties in crystallising RNA:protein complexes. The lack of complex structures results in poorly understood RNA recognition patterns and methylation reaction mechanisms. On the contrary, many cofactor-bound MTase structures are available, resulting in well-understood protein:cofactor recognition, that can guide the design of bisubstrate analogues that mimic the state at which both the substrate and the cofactor is bound. Such bisubstrate analogues were recently synthesized for proteins monomethylating the N6-atom of adenine (m6A). These proteins include, amongst others, RlmJ in E. coli and METLL3:METT14 and METTL16 in human. As a proof-of-concept, we here test the ability of the bisubstrate analogues to mimic the substrate:cofactor bound state during catalysis by studying their binding to RlmJ using differential scanning fluorimetry, isothermal titration calorimetry and X-ray crystallography. We find that the methylated adenine base binds in the correct pocket, and thus these analogues could potentially be used broadly to study the RNA recognition and catalytic mechanism of m6A MTases. Two bisubstrate analogues bind RlmJ with micro-molar affinity, and could serve as starting scaffolds for inhibitor design against m6A RNA MTases. The same analogues cause changes in the melting temperature of the m1A RNA MTase, TrmK, indicating non-selective protein:compound complex formation. Thus, optimization of these molecular scaffolds for m6A RNA MTase inhibition should aim to increase selectivity, as well as affinity. P. Asadi-Atoi, P. Barraud, C. Tisné and S. Kellner*, Benefits of stable isotope labeling in RNA analysis.
Abstract:
RNAs are key players in life as they connect the genetic code (DNA) with all cellular processes dominated by proteins. They contain a variety of chemical modifications and many RNAs fold into complex structures. Here, we review recent progress in the analysis of RNA modification and structure on the basis of stable isotope labeling techniques. Mass spectrometry (MS) and NMR spectroscopy are the key tools and many break-through developments were made possible by analysis of stable isotope labeled RNA. Therefore, we discuss current stable isotope labeling techniques such as metabolic labeling, enzymatic labeling and chemical synthesis. RNA structure analysis by NMR is challenging due to two major problems that become even more salient when the size of the RNA increases, namely chemical shift overlaps and line broadening leading to complete signal loss. Several isotope labeling strategies have been developed to provide solutions to these major issues, such as deuteration, segmental isotope labeling or site-specific labeling. Quantification of modified nucleosides in RNA by mass spectrometry is only possible through the application of stable isotope labeled internal standard. With nucleic acid isotope labeling coupled mass spectrometry, NAIL-MS, it is now possible to analyze the dynamic processes of post-transcriptional RNA modification and demodification. The trend, in both NMR and MS RNA analytics, is without doubt shifting from the analysis of snapshot moments towards the development and application of tools capable of analyzing the dynamics of RNA structure and modification profiles. C. Bou-Nader, P. Barraud, L. Pecqueur, S. Sacquin-Mora, J. Pérez, C. Velours, M. Fontecave, C. Tisné & D. Hamdane*, Molecular basis for transfer RNA substrate recognition by human dihydrouridine synthase.
Abstract:
Double stranded RNA-binding domain (dsRBD) is a ubiquitous domain specialized in the recognition of double-stranded RNAs (dsRNAs). Present in many proteins and enzymes involved in various functional roles of RNA metabolism, including RNA splicing, editing, and transport, dsRBD generally binds to RNAs that lack complex structures. However, this be- lief has recently been challenged by the discovery of a dsRBD serving as a major tRNA binding module for human dihydrouridine synthase 2 (hDus2), a flavoenzyme that catalyzes synthesis of dihydrouridine within the complex elbow structure of tRNA. We here unveil the molecular mechanism by which hDus2 dsRBD recognizes a tRNA ligand. By solving the crystal structure of this dsRBD in complex with a dsRNA together with extensive characterizations of its interaction with tRNA using mutagenesis, NMR and SAXS, we establish that while hDus2 dsRBD re- tains a conventional dsRNA recognition capability, the presence of an N-terminal extension appended to the canonical domain provides additional residues for binding tRNA in a structure-specific mode of action. Our results support that this extension represents a feature by which the dsRBD specializes in tRNA biology and more broadly highlight the importance of structural appendages to canonical domains in promoting the emergence of functional diversity.
Abstract:
A. Mouhand§, A. Belfetmi§, M. Catala, V. Larue, L. Zargarian, F. Brachet, R. J. Gorelick, C. Van Heijenoort, G. Mirambeau, P. Barraud, O. Mauffret & C. Tisné*, Modulation of the HIV nucleocapsid dynamics finely tunes its RNA-binding properties during virion genesis. During HIV-1 assembly and budding, Gag protein, in particular the C-terminal domain containing the nucleocapsid domain (NCd), p1 and p6, is the site of numerous interactions with viral and cellular factors. Most in vitro studies of Gag have used constructs lacking p1 and p6. Here, using NMR spectroscopy, we show that the p1–p6 region of Gag (NCp15) is largely disordered, but interacts transiently with the NCd. These interactions modify the dynamic properties of the NCd. Indeed, using isothermal titration calorimetry (ITC), we have measured a higher entropic penalty to RNA-binding for the NCd precursor, NCp15, than for the mature form, NCp7, which lacks p1 and p6. We propose that during assembly and budding of virions, concomitant with Gag oligomerization, transient interactions between NCd and p1– p6 become salient and responsible for (i) a higher level of structuration of p6, which favours recruitment of budding partners; and (ii) a higher entropic penalty to RNA-binding at specific sites that favours non-specific binding of NCd at multiple sites on the genomic RNA (gRNA). The contributions of p6 and p1 are sequentially removed via proteolysis during Gag maturation such that the RNA-binding specificity of the mature protein is governed by the properties of NCd.
Abstract:
S. E. El Meshri, E. Boutant, A. Mouhand, A. Thomas, V. Larue, L. Richert, V. Vivet-Boudout, Y. Mély, C. Tisné*, D. Muriaux* & H. de Rocquigny*, The NC domain of HIV-1 Gag contributes to the interaction of Gag with TSG101. Background: HIV-1 Gag polyprotein orchestrates the assembly of viral particles. Its C-terminus consists of the nucleocapsid (NC) domain that interacts with RNA, and the p6 domain containing the PTAP motif that binds the cellular ESCRT factor TSG101 and ALIX. Deletion of the NC domain of Gag (GagNC) results in defective Gag assembly, a decrease in virus production and, thus probably affects recruitment of the ESCRT machinery. To investigate the role of GagNC in this recruitment, we analysed its impact on TSG101 and ALIX localisations and interactions in cells expressing Gag.
Abstract:
Methods: Cells expressing mCherry-Gag or derivatives, alone or together with eGFP-TSG101 or eGFP-ALIX, were analysed by confocal microscopy and FLIM-FRET. Chemical shift mapping between TSG101-UEV motif and Gag C-terminus was performed by NMR.
Results: We show that deletion of NC or of its two zinc fingers decreases the amount of Gag-TSG101 interacting complexes in cells. These findings are supported by NMR data showing chemical shift perturbations in the NC domain in- and outside - of the zinc finger elements upon TSG101 binding. The NMR data further identify a large stretch of amino acids within the p6 domain directly interacting with TSG101.
Conclusion: The NC zinc fingers and p6 domain of Gag participate in the formation of the Gag-TSG101 complex and in its cellular localisation.
General significance: This study illustrates that the NC and p6 domains cooperate in the interaction with TSG101 during HIV-1 budding. In addition, details on the Gag-TSG101 complex were obtained by combining two high resolution biophysical techniques.